Modern marvels.
Of all the science headlines of the 1920s, “Ice Cream from Crude Oil” may best capture the era’s unbridled enthusiasm for chemistry. Edwin E. Slosson, the first head of the news service that would become Science News, reported that fats can be made by breaking up and rearranging the molecules of mineral oil. Synthetic ice cream was just one of the wonders that could lie around the corner.
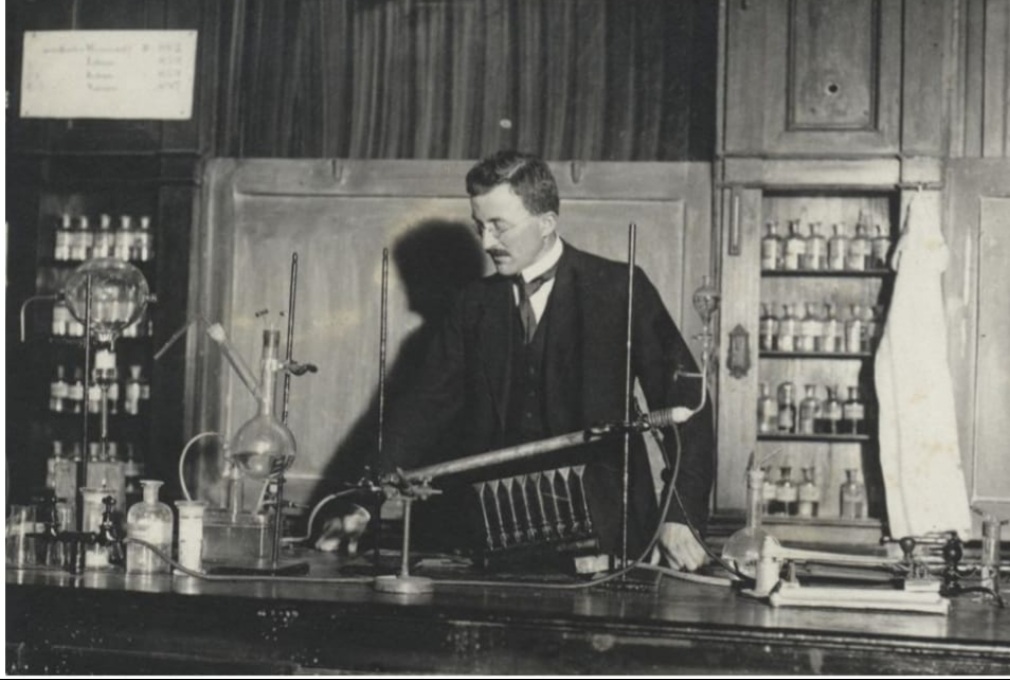
In the 1920’s German chemist Hermann Staudinger showed that small molecules can link up in chains to form very large molecules. The discovery helped set the stage for a boom in synthetic materials, including plastics.ETH-BIBLIOTHEK ZÜRICH, BILDARCHIV, FOTOGRAF: UNBEKANNT, PORTR_14413-016-AL, PUBLIC DOMAIN MARK.
Slosson went on to describe the potential to create aromatics, flavorings, nitroglycerine for dynamite, plastics, drugs and dyes “of infinite variety.” Petroleum would become increasingly valuable “as a source of substances for which man has hitherto been dependent upon the chance bounty of nature.”
Petroleum-based ice creams never became the new it thing, yet Slosson’s reporting was on point. The last century has witnessed a dramatic leap in the ability to study and synthesize matter. From our homes and cities to our electronics and clothing, much of what we interact with every day is made possible through the manipulation, recombination and reimagination of the basic substances nature has provided.
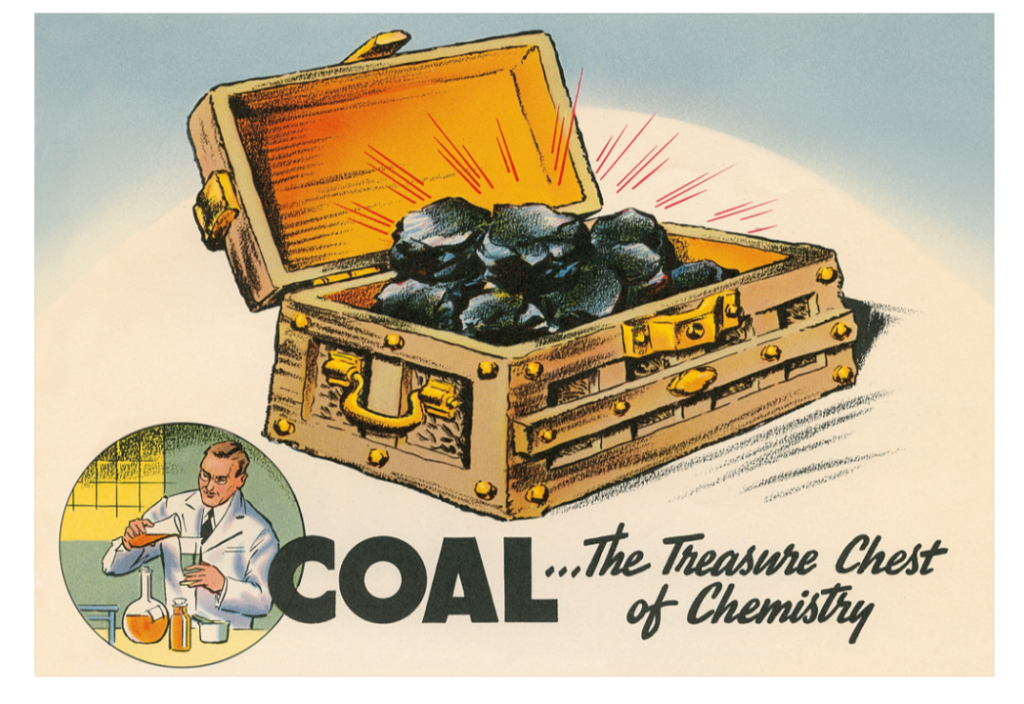
FOUND IMAGE HOLDINGS/CORBIS VIA GETTY IMAGES
“The world is unrecognizable from 100 years ago,” says Anna Ploszajski, a materials scientist and author of Handmade: A Scientist’s Search for Meaning Through Making. And that, she says, is “simply because of the materials that we have around, let alone all of the new ways we use them.”
The turn of the 20th century set the stage for a new era of materials. Organic chemists learned how to turn coal into a variety of industrial chemicals, including dyes and perfumes. Later, motivated by wartime demand, chemists honed their craft with poison gas, explosives and propellants, as well as disinfectants and antiseptics —so many new products that World War I was often called “the chemist’s war.” And at a fundamental level, the new century also ushered in greater understanding of chemical bonds and the atom, its constituents and its behavior.
In the decades that followed, approaches in chemistry and physics combined with engineering to give rise to a new field, now called materials science. A boost from the U.S. government, which was seeking new materials for space and military technologies during the Cold War, led to academic departments that brought together physicists, chemists, metallurgists and electrical engineers, anyone interested in materials.
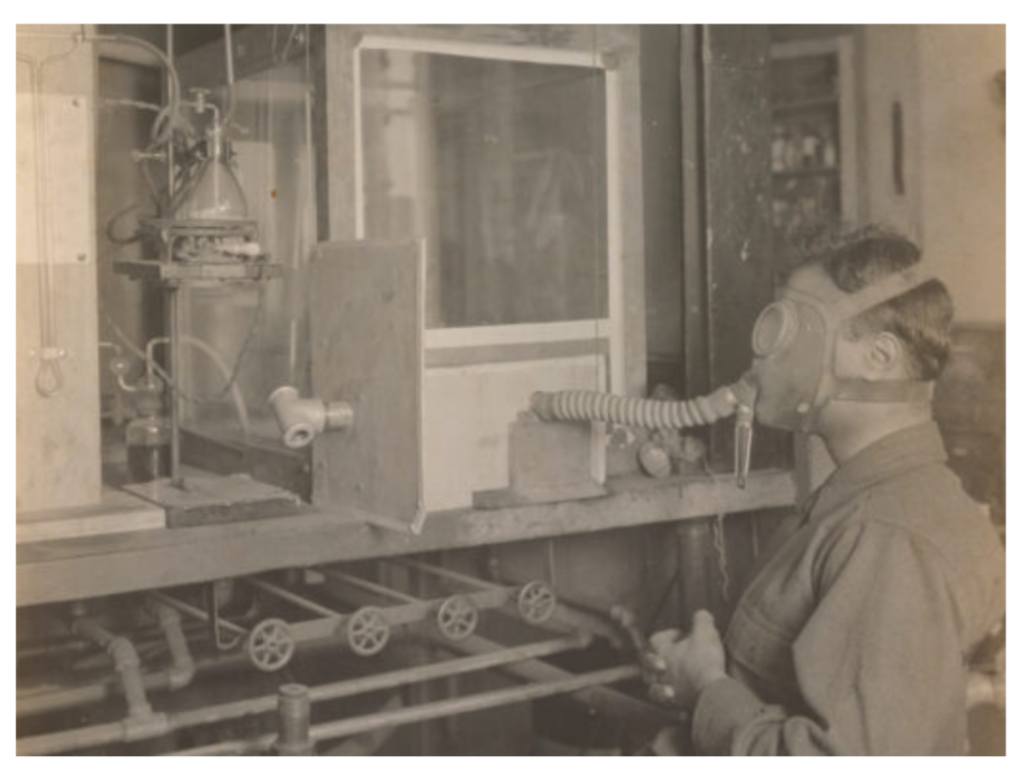
NATIONAL ARCHIVES #86714735
An extensive survey of the field, put together by the National Academy of Sciences in the 1970s and titled Materials and Man’s Needs, defined materials as “substances having properties which make them useful in machines, structures, devices and products.” Here’s how the report described the pace of research.
“The transitions from, say, stone to bronze and from bronze to iron were revolutionary in impact, but they were relatively slow in terms of the time scale. The changes in materials innovation and application within the last half century occur in a time span which is revolutionary rather than evolutionary.”
Alongside this new science came new and improved scientific tools.
Scientists can now see materials at a much finer scale, with the electron microscope making individual atoms visible. X-ray crystallography unveils atomic arrangements, allowing for a better understanding of materials’ structure. And equipment such as chromatographs and mass spectrometers let today’s scientists untangle mixtures of chemicals and identify the compounds within. Francis Aston first took advantage of a mass spectrometer in his study of isotopes in 1919, but for a long time the tool was seen by some chemists as, according to a description by mass spectrometrist Michael Grayson, “an unexplainable, voodoo, black magic kind of a tool.”
Many new materials were birthed from basic curiosity and serendipity. But new techniques also made way for targeted innovation.
Today materials can be designed from scratch to solve specific problems. And explorations of the properties of solid substances — for instance, how matter interacts with heat, light, electricity or magnetism — along with iterations of design have further contributed to the stuff that surrounds us, giving way to transistors, transition lenses, touch screens and hard disk drives.
Explorations into how matter interacts with biological tissues have yielded coronary stents, artificial skin and also hip replacements, including metal mélanges that are tough and nonreactive when they sit against bone.
“Materials science and engineering over the millennia have gone from ‘What do you find outside the cave?’ to the beginnings of materials-by-design,” Stephen H. Carr of Northwestern University told Science News in the 1980s.
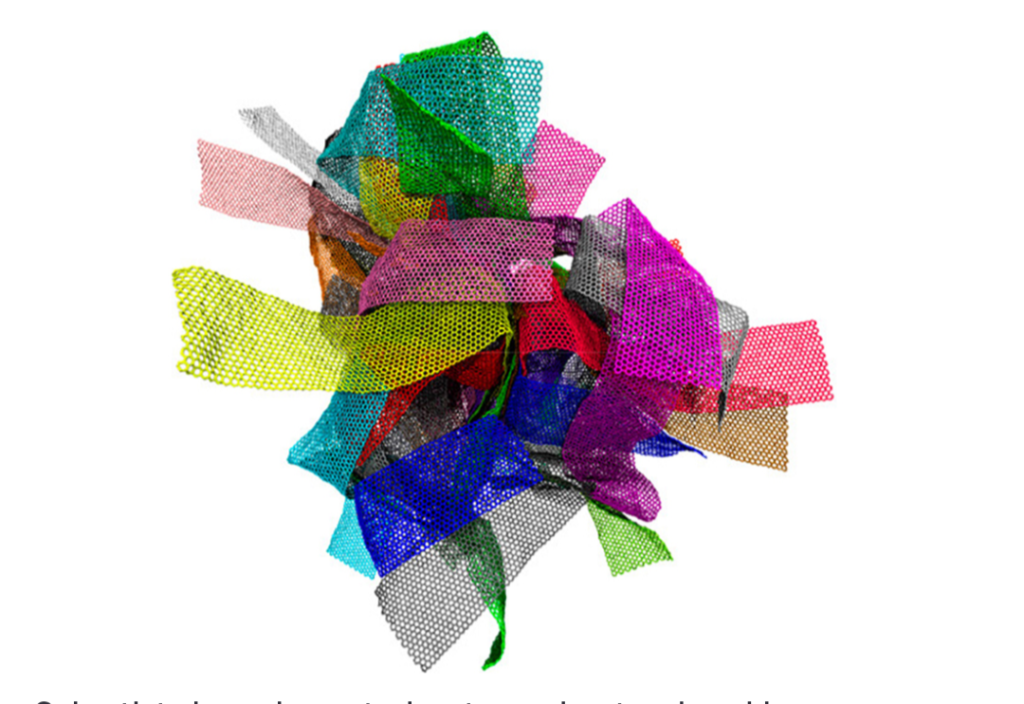
Scientists have been trying to understand and harness graphene’s behavior since its discovery in 2004. Simulations by researchers at the National Institute of Standards and Technology, for example, show that melted sheets (each a different color) crumple into a foamy material that could prove useful for sensors, filters and insulators.
XIA WENJIE, RIDVAN KAHRAMAN/NORTH DAKOTA STATE UNIVERSITY.
The outputs of such efforts are all around us. Take air travel, for example, and the global interconnectivity it introduced. It’s possible thanks to alloys that are lightweight and robust. And today’s personal connectivity — via smartphones and computers — came with transistors made of silicon.
Their small size and low power requirements brought computing to work, and then into our homes and pockets. An abundance of plastic housewares and comfy athleisure options are made possible via improvements in polymers.
The examples below highlight just a handful of ways that advances in materials over the last century have transformed our daily lives — we’ve focused on travel, connectivity and convenience. We could have chosen countless others.
Yet innovation hasn’t come without consequences. For each tale of progress, there are also stories of the marks people have left on this planet. While enabling humans to flourish, many new substances have become pollutants, from PCBs to plastics. However people go about addressing these environmental problems, other new materials will inevitably play a role in the solutions.
Carolyn Wilke.
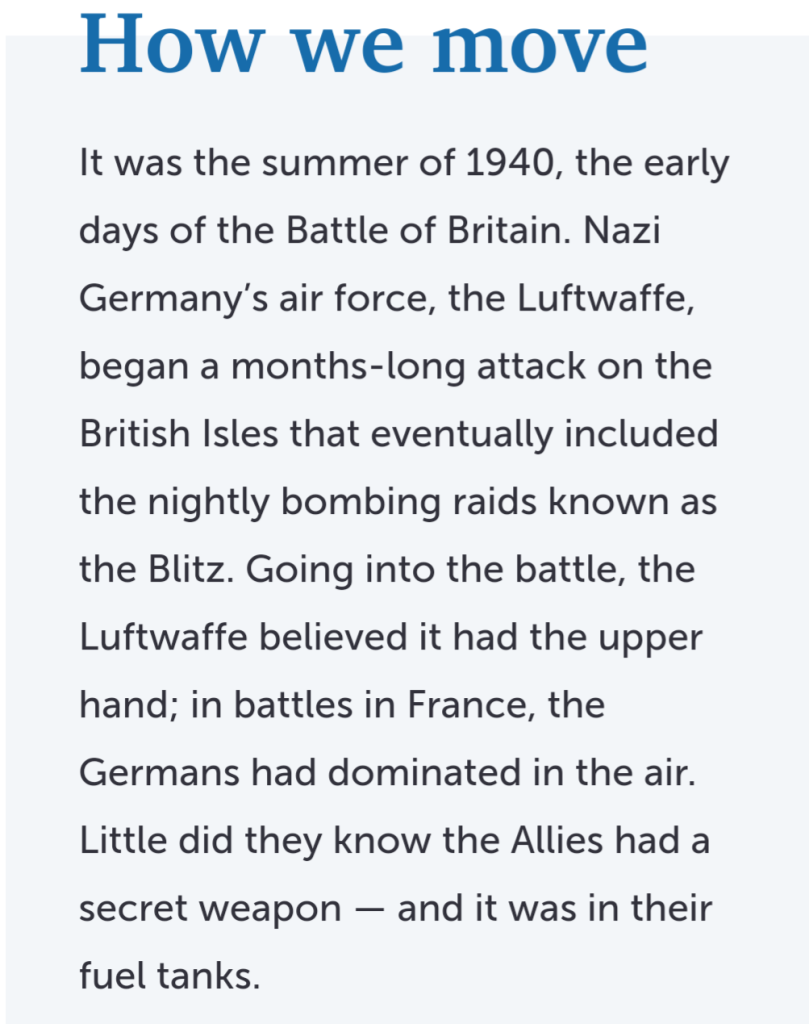
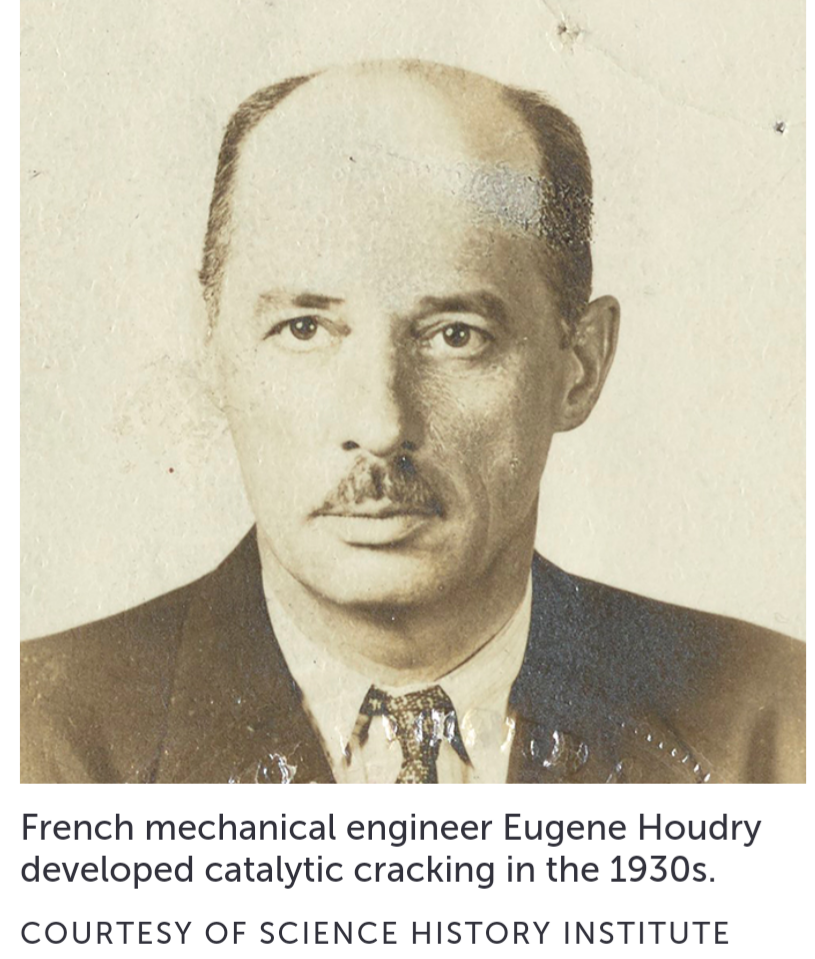
As Germans began flying over England, they were surprised to find the tables had turned. One important factor was not the planes themselves, but the octane rating of the fuel they ran on. The British Spitfires and Hurricanes that the Germans had outmaneuvered in France could now climb higher and fly faster thanks to fuel made with a newly developed process called catalytic cracking.
Catalysts boost chemical reactions by reducing the energy needed to get them going. French mechanical engineer Eugene Houdry had developed a catalytic process in the late 1930s to make high-octane fuel, which can withstand higher compression and allows engines to deliver more power.
Simply increasing the octane rating of aviation fuel from 87 to 100 gave the Allies a crucial edge.
Houdry wasn’t the first to attempt to use catalysts to bust the big molecules of heavy fuels into smaller ones to improve performance. But as an avid road racer, he had a special interest in high-quality gasoline. He studied hundreds of catalysts until he landed on aluminum- and silicon-based materials that could do the busting more efficiently than an existing process that relied on heat. When he tested his gasoline in his Bugatti racer, he reached speeds of 90 miles per hour.
Shortly after catalytic cracking was first demonstrated at industrial scale in 1937, Science News Letter, the predecessor of Science News, described it as “a radically new refining process held to be of the greatest importance to the entire petroleum industry.” By then Houdry had moved to the United States and joined Socony-Vacuum Oil and Sun Oil to build pilot plants. The first large-scale “Houdry unit” began operating at Sun Oil’s Marcus Hook refinery in Pennsylvania, and units multiplied from there.
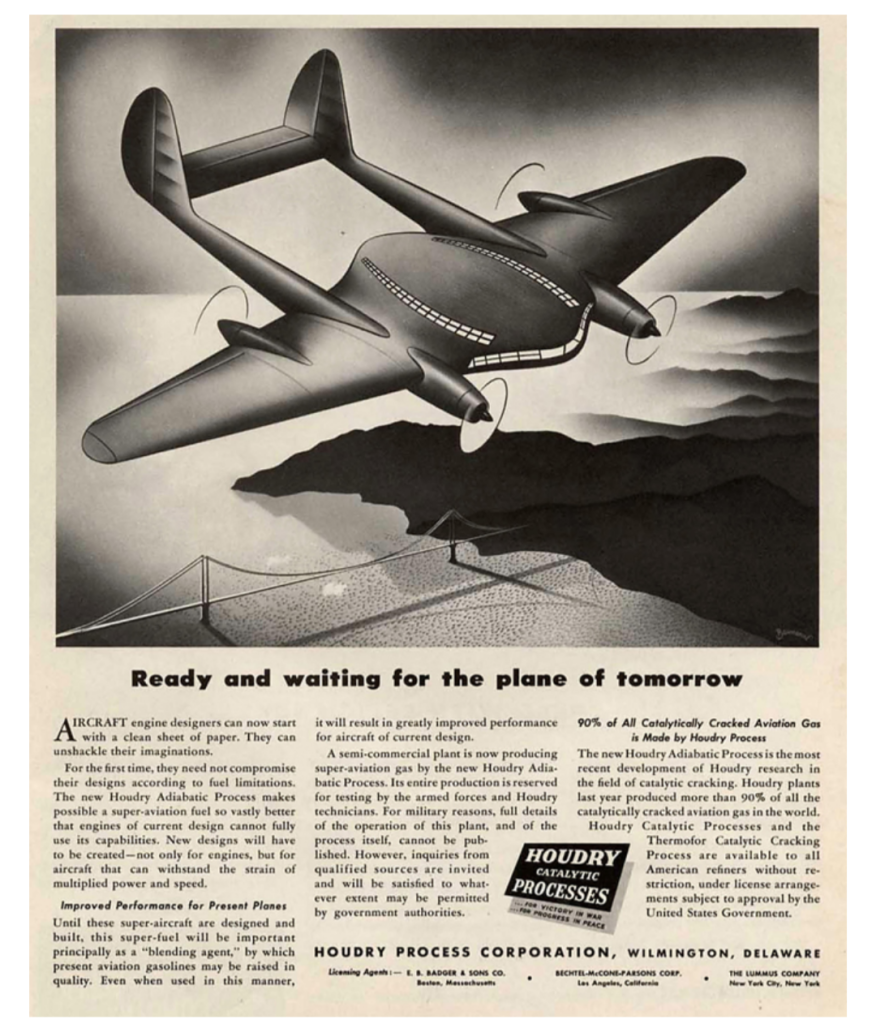
RETRO ADARCHIVES/ALAMY STOCK PHOTO.
In the following decades, catalytic cracking and improvements to the process Houdry pioneered would contribute to the reign of automobiles, and catalytic cracking still produces much of the gas that cars guzzle today.
But all that driving soon began to take a toll on the environment.
When the hydrocarbon molecules in gasoline burn, the engine exhaust contains small amounts of harmful gases: poisonous carbon monoxide, nitrogen oxide that can cause smog and acid rain, as well as unburned hydrocarbons. With so many vehicles hitting the road, cities such as Los Angeles choked on smog in the 1940s and ’50s.
Houdry, after helping to fuel those vehicles, looked again to catalysts to deal with the pollution that internal combustion engines caused. He designed a catalytic converter.
“When first considered, the problem seems simple,” Houdry wrote in a 1954 patent application. “A great number of catalysts can be used for the reaction. By simply placing one of these catalysts in the exhaust line under controlled conditions, the exhaust fumes can be cleaned.” The catalysts, precious metals such as platinum or palladium, provide docking sites for the harmful gases to hang onto; there, reactions involving oxygen convert them to less harmful forms. But, as Houdry pointed out, the high temperatures, mechanical shock and other conditions in an engine complicate the problem.
In the 1950s, Houdry set out his solution, outlining a series of reactions, materials and conditions necessary for a working catalytic converter. But he was ahead of his time. For years, the adoption of catalytic converters in automobiles was stymied by leaded gasoline, which improved octane ratings but gummed up the catalysts’ surfaces. Within a few decades of the passage of the Clean Air Act of 1970, which led to requirements for catalytic converters and lead-free fuel, the devices helped clear the air in cities around the world.
Taking to the air.
Air travel for the masses encountered a different dilemma; lightening the load. The earliest airplanes gained lift at the turn of the 20th century on wings of fabric and wood, but to really soar, airplanes needed light but strong materials. The first aircraft designed for passengers, the Ford Trimotor — nicknamed the Tin Goose — took to the air in 1926 with help from aluminum alloys.
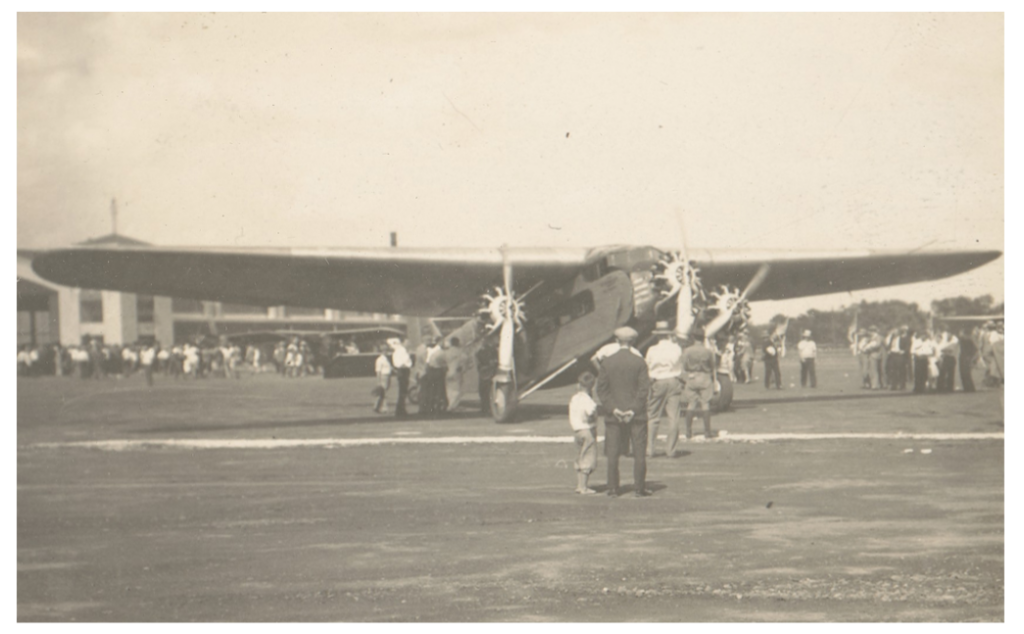
GIFT OF WESTON J. NAEF, IN MEMORY OF KATHLEEN W. NAEF AND WESTON J. NAEF SR. 1982/WIKIMEDIA COMMONS.
Alloys have existed since ancient times. Bronze Age artisans combined copper with arsenic or tin in crucibles to make tools, jewelry and more. From there, advances coincided with the ability to melt metals at higher and higher temperatures, eventually leading to steel. Scientists since have studied how materials’ structures and properties — including desirable features like strength, bendability and resistance to corrosion — vary with composition, temperature and processing.
The fuselage of the Tin Goose contained a newly developed alloy named duralumin, a contraction of “Dürener” (for the company that originally made it) and “aluminum.” Developed by Alfred Wilm, a German metallurgist seeking a lighter alloy that could replace brass in ammunition cartridges, duralumin combined aluminum with copper, magnesium and manganese and got its strength from a series of heat treatments.
After adjusting the amount of magnesium in his mixture one day in 1906, Wilm left for the weekend, charging his assistant to test the hardness again on Monday. To their surprise, they discovered a new, never-before-seen feature of some alloys: Simply sitting at room temperature, the material had gotten harder as it sat.
In 1926, Science News Letter described the promise of materials such as duralumin for safer dirigibles, which would carry large numbers of passengers into the air: “Of these sound materials, strong and light girders must be built. So light that a man can carry one of them in his hand and yet so strong that they will carry loads of thousands of pounds.” The rigid frame of the Hindenburg, for example, was made of duralumin.
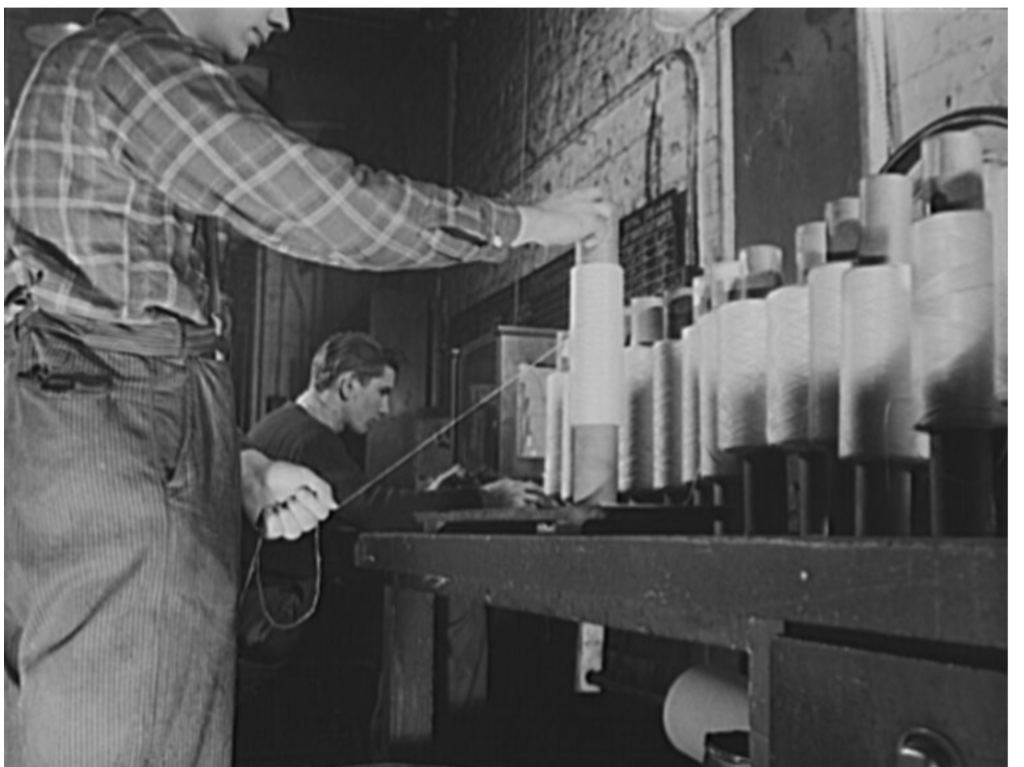
ALFRED T PALMER/UNITED STATES OFFICE FOR EMERGENCY MANAGEMENT.
But dirigibles and duralumin were just the beginning. The 20th century has seen an explosion in types of alloys and their applications, from stainless steel cutlery to the titanium alloys used in prosthetics and pacemakers to crucial components of vehicles. Today’s jet engines are built of superalloys, which can withstand infernal temperatures.
Plastics and composites have also helped planes shed weight. While alloys melt metals and sometimes other types of elements together, composites combine materials with very different properties — such as glass and plastic — by suspending one in the other or sandwiching them together, for instance. Because they can be tuned to be light and strong, composites have made their way into parts all over planes, from the engine to the wings. Boeing’s 787 Dreamliner, which debuted in 2007, is made up of 50 percent composites by weight.
Composites also show up
all over today’s cars, from dashboards to bumpers, with various materials glued together to better protect driver and passengers. “The incredible use of adhesives in cars to create crumple zones so that the passenger compartment is safer,” for example, is “directly out of materials,” says Mark Jones, a chemist and member of the National Historic Chemical Landmarks committee of the American Chemical Society.
And to Space.
When the Apollo 11 astronauts reentered Earth’s atmosphere after their historic moonwalk in 1969, the outer surface of their module skyrocketed to somewhere around 5,000° Fahrenheit, hot enough to incinerate any living thing. But inside, the astronauts sat at comfortable temperature around 70° F or so, protected by a heat shield made of a material called Avcoat.
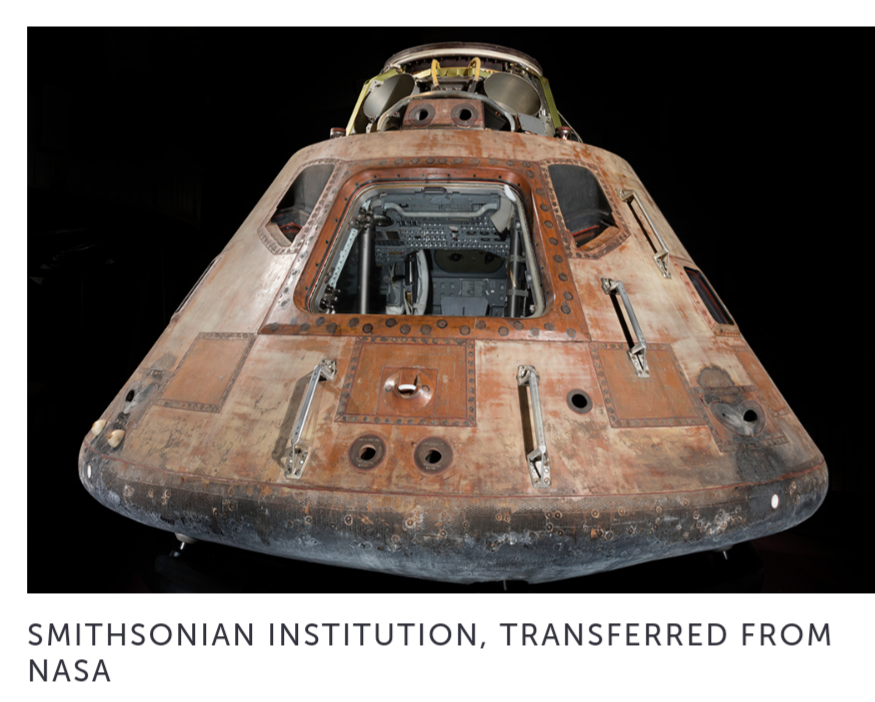
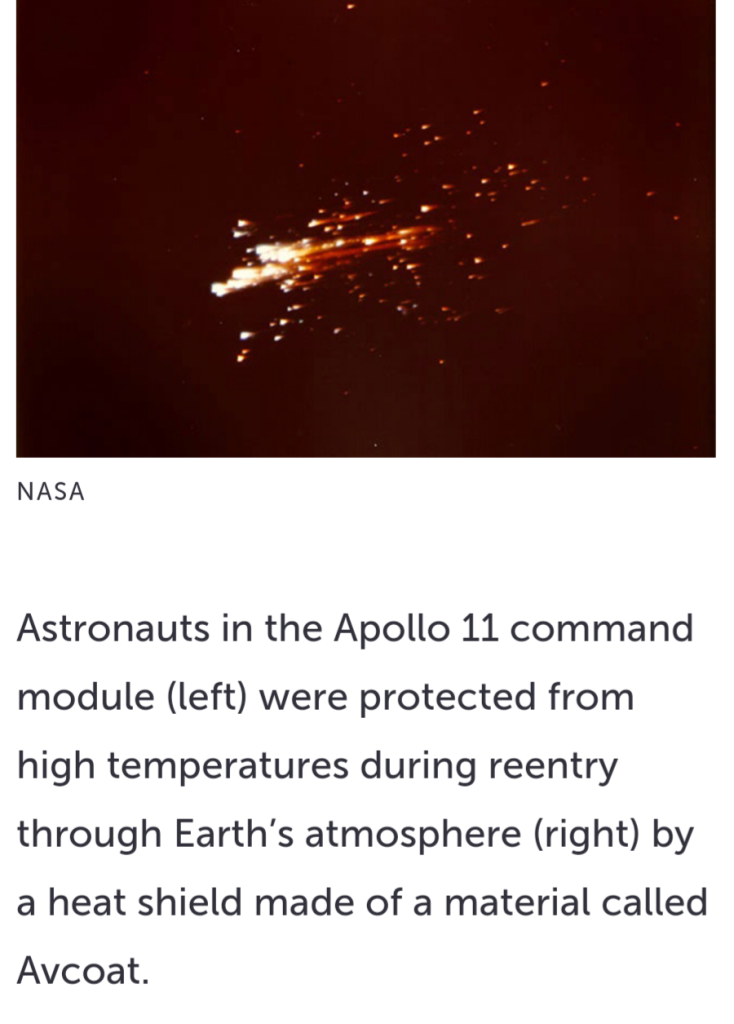
Avcoat is a type of reinforced plastic, made from an epoxy resin filling a honeycomb fiberglass network. A layer of it, which varied in thickness from 0.5 to 3 inches, dissipated heat away from the command module’s metal components, keeping temperatures inside from rising.
Research advances that led to alloys and composites, as well as plastics — many of which ended up in cars and planes — got a boost from a desire to get to space. Spacefaring vehicles have to be not only strong and lightweight, but also withstand extreme temperatures.
A new NASA spaceship called Orion, destined to take people to the moon and beyond, again uses Avcoat.
But engineers have come up with a far more efficient process to make it. Instead of injecting Avcoat individually into each fiberglass cell, the Avcoat material is now made in pieces, which are machined to size before they are bonded onto a heat shield more than 16 feet in diameter. An Orion test mission to the moon could launch in early 2022.
Space materials push composites to the max. One extreme composite is Nextel.
Sheets of it, woven of the ceramic fiber and Kevlar, another materials innovation, protect the International Space Station from debris and meteoroids. In other cases, materials invented for air and space travel have stuck around on Earth. Memory foam, developed in the late 1960s to cushion pilots and passengers during takeoff and landing, is now better known for its use in mattresses. And space blankets, which arrived that same decade to protect electronics from drastic swings of temperature, show up in emergency kits and are donned by marathoners after a race.
Carolyn Wilke.
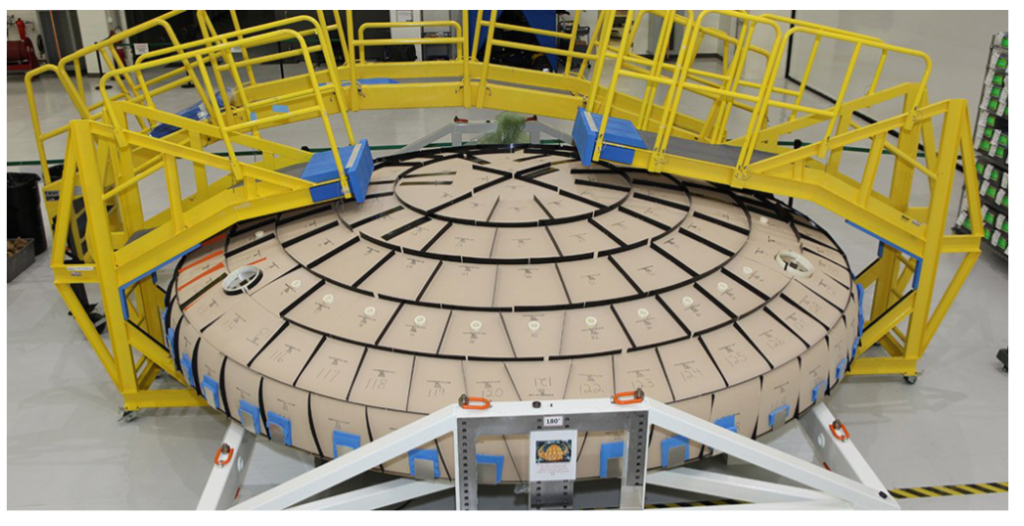
NASA.
Courtesy and source century of science by science news.org